Scientific description of Phytophthora (Late Blight) (2020)
Based on Adolf B. et al. (2020) Fungal, Oomycete, and Plasmodiophorid Diseases of Potato. In: Campos H., Ortiz O. (eds) The Potato Crop. Springer, ChamThe authors of this content are Hannele Lindqvist-Kreuze, Willmer Perez, Peter Kromann and Jorge Andrade-Piedra
Late blight is caused by Phytophthora infestans (Mont.) de Bary. It was previously classified as a fungus due to the superficial resemblance to filamentous fungi but is now classified as oomycete in the kingdom of stramenopiles (Kamoun et al. 2014). The vegetative stage of the mycelium in P. infestans is diploid, while in true fungi it is haploid.
However, recent research has shown that in the modern-day lineages the progenies from sexual P. infestans populations are diploid, but the most important pandemic clonal lineages are triploid (Li et al. 2017). Virulence of oomycetes depends on large, rapidly evolving protein families including extracellular toxins, hydrolytic enzymes, and cell entering effectors that help the pathogen suppress the host plant defenses and gain nutrition from the host (Jiang and Tyler 2012).
Elicitins are an example of structurally conserved extracellular proteins of P. infestans that have a function in the sequestration of sterols from the host plant, but can also act as pathogen-associated molecular patterns (PAMPs), and as such can activate PAMP triggered immunity (PTI) (Du et al. 2015).
P. infestans secretes large numbers of effectors: apoplastic effectors, such as EPIC1, interact with the host cell wall, host proteases and other defense-related molecules in the host extracellular space, while cytoplasmic effectors, the RxLR proteins, and CRNs (crinkling and necrosis-induced proteins) function inside the plant cells (reviewed in Whisson et al. 2016).
RxLR effectors act as activators of plant immunity, resulting in effector triggered immunity (ETI) (Oh et al. 2009; Wang et al. 2017a), while the apoplastic effectors, similarly to elicitins, act as activators of the PTI (Domazakis et al. 2017). Recent research has also shown that some P. infestans effectors can target host proteins whose activity enhances susceptibility possibly through the inhibition of positive regulators of immunity or promote the activity of susceptibility (S), that in turn can negatively regulate immunity (Boevink et al. 2016).
The effector genes locate mostly in the gene sparse regions of the genome, that are rich in repetitive sequences and are rapidly evolving, probably enabling the evolutionary arms race between P. infestans and the host plant (Haas et al. 2009; Dong et al. 2015).
Symptoms
The asexual, aerially dispersed sporangia (see illustration below) are responsible for most of the devastating epidemics on potato. When the sporangia lands on a plant surface it can germinate directly or first form zoospores, which encyst, germinate, and penetrate the host tissue (reviewed by Fry et al. 2015).
This stage of infection is unnoticeable to the naked eye, but inside the plant cell a repertoire of molecular interactions takes place. After penetration and adhesion, the pathogen forms haustoria inside the plant cells, from where it secretes effector proteins (reviewed in Nowicki et al. 2012; Whisson et al. 2016; Wang et al. 2017a). At this biotrophic stage P. infestans requires living cells to obtain nutrients.
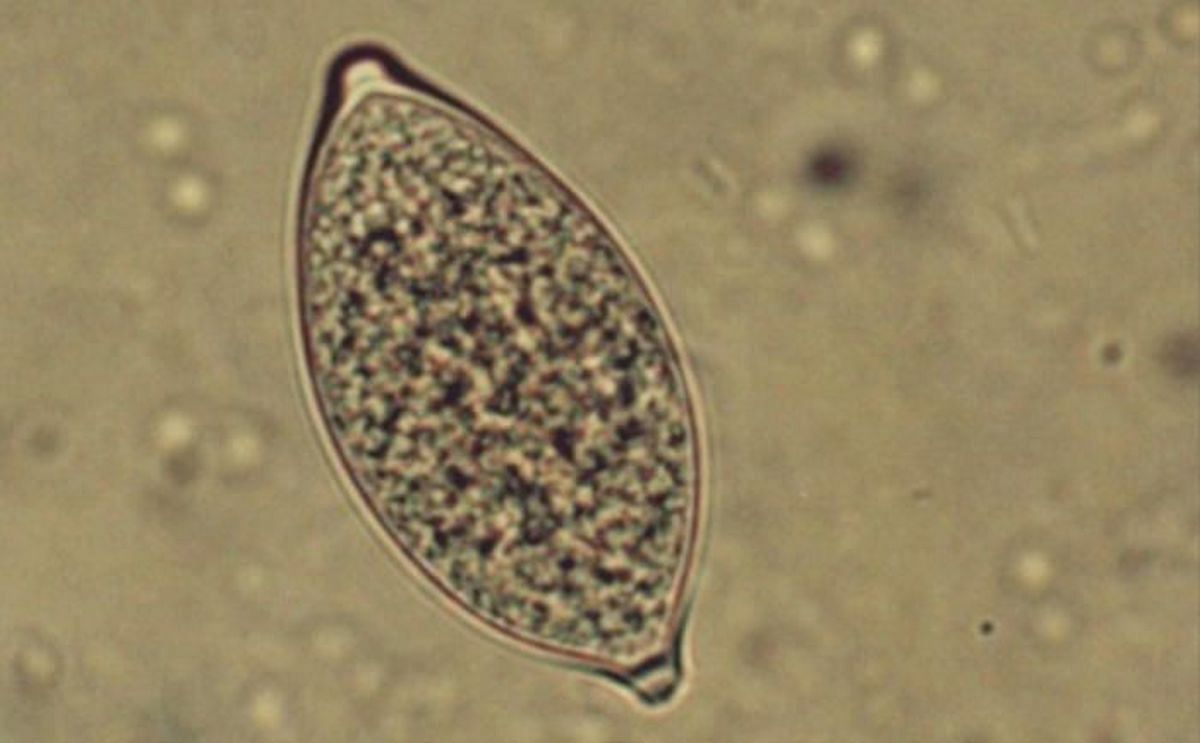
Lemon-shaped sporangium of Phytophthora infestans
Symptoms typically begin to develop where water accumulates near the leaf edges or tips and in stems near petioles.
Affected tubers show irregular, slightly depressed areas with brown color. In a cross-section, finger-like extensions can be seen from the external surface to the tuber medulla (Perez and Forbes 2010).
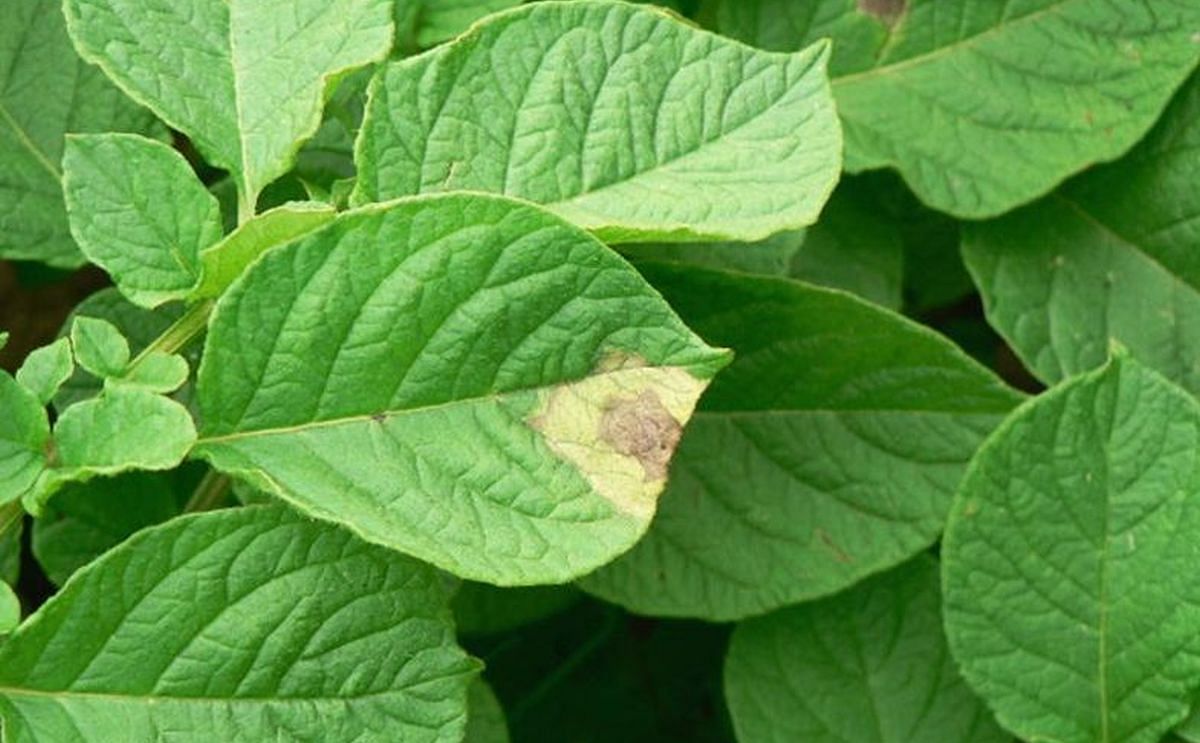
Symptoms of late blight on leaves
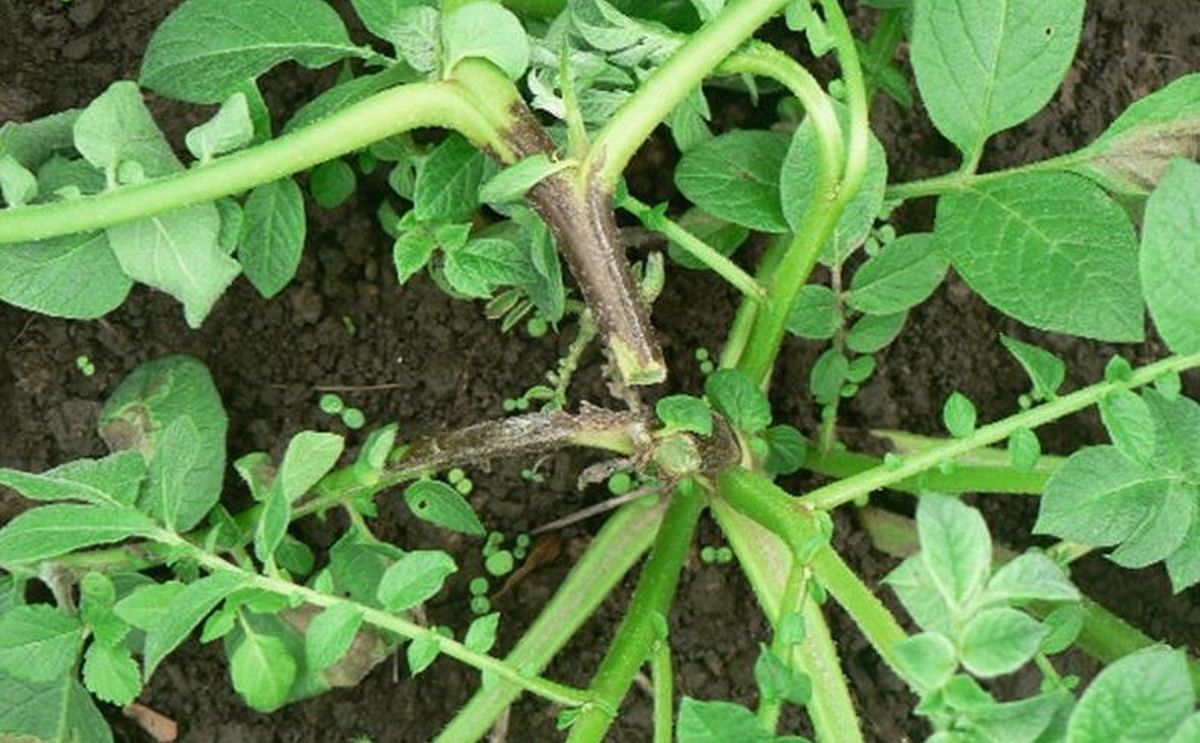
Symptoms of late blight on stems.
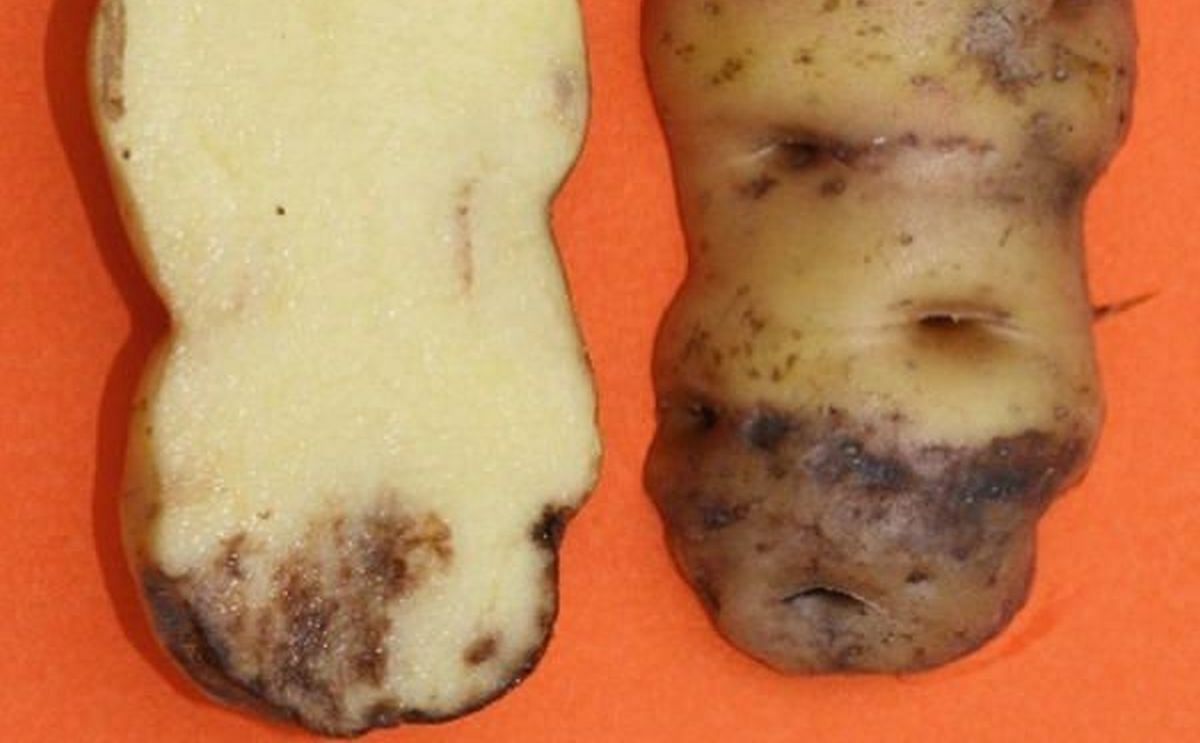
External and internal symptoms of late blight in tubers
During spore formation and germination, large numbers of genes involved in pathogenesis, calcium signaling, and metabolism are upregulated or transcribed in waves (Ah-Fong et al. 2017), whereas genes of the fatty acid biosynthesis pathway are downregulated (Rodenburg et al. 2018).
Identification of the proteins and enzymes essential for pathogen growth and development, and linked to the different symptomatic reactions in the plant, can lead to the discovery of potential targets for crop protection chemicals.
High level of moisture is essential for the lesion development, and under optimal conditions the disease can advance very fast and destroy the plant in matter of days (Perez and Forbes 2010).
Impact
The potential economic and social impact of potato late blight is best illustrated by the well-publicized role it played in the Irish Famine in the middle of the nineteenth century. Because of the famine, millions of Irish died or emigrated (Bourke 1993).
Other devastating late blight outbreaks have been reported around the world, causing food insecurity, hunger (International Potato Center 2007), and oftentimes crippling the local potato industry.
Haverkort et al. (2009) estimated that the global costs and losses due to late blight may take 16% of all global potato production. At 100 €/t the world potato production represents a value of €38 billion today.
The 16% loss then represents an annual financial loss of €6.1 billion per annum today, considering that the increase in global potato production in the last decade has mainly been in developing countries, which suffer low yields and the vast majority of these estimated losses to late blight compared to developed countries.
Resistance to Late Blight
After the discovery of the Mexican wild species Solanum demissum as an excellent source of resistance, eleven major genes were introduced in cultivated tetraploid potato breeding lines (Black et al. 1953; Malcolmson and Black 1966). Although some of these genes can be considered defeated, others, for example R8, are still effective against current pathogen populations (Vossen et al. 2016).
Over 50 R genes have been identified from wild Solanum species as detailed by Rodewald and Trognitz (2013), and the research field remains active with a growing list of genes available for potato breeding programs (Jo et al. 2015; Vossen et al. 2016; Witek et al. 2016; Yang et al. 2017).
However, due to crossing barriers and linkage drag, there are only few successful cases where R genes have been introduced into improved tetraploid breeding lines by classical breeding (Bethke et al. 2017).
Introduction of a single R gene from wild germplasm is a lengthy procedure as demonstrated by the examples of commercial varieties Bionica and Toluca that contain Rpi-blb2 originating from S. bulbocastanum, and were released almost 50 years after the first crosses were made (Haverkort et al. 2016).
Genetic engineering bears promise and varieties containing stacked or single R genes are in the process of being released in the markets that accept this technology (Haverkort et al. 2016; Schiek et al. 2016; Pacifico and Paris 2016; Ghislain et al. 2018). As opposed to the major R genes, quantitative resistance was generally expected to be governed by many minor genes.
However, recently it was shown that R genes can also have quantitative effects. The potato cultivar Sarpo Mira contains at least four R genes that confer complete resistance against incompatible isolates and a quantitative R gene, Rpi-Smira1 that confers broad-spectrum field resistance (Rietman et al. 2012).
A biparental cross using a haploidized resistant clone from the CIP B3 population was used to locate a strong QTL in chromosome 9 (Li et al. 2012a). Subsequent association mapping confirmed the importance of the same genome region for late blight resistance in the tetraploid B3 breeding population (Lindqvist-Kreuze et al. 2014), and recently the R8 gene was identified in the QTL by dRenseq (Jiang et al. 2018).
Identification of new resistance sources and functional resistance or susceptibility genes has been recently greatly accelerated by modern techniques, such as effectoromics and resistance gene enrichment sequencing technologies. To date, all effector proteins identified that are recognized by the plant resistance (R) proteins belong to the RXLR category.
Therefore, RXLR effectors cloned into expression vectors have been used to successfully identify functional new R genes from potato germplasm using agroinfection (Vleeshouwers et al. 2011; Vleeshouwers and Oliver 2014). Apoplastic effectors are recognized by pathogen recognition receptors (PRR) and can be used in a similar manner to identify resistance germplasm (Domazakis et al. 2017).
In the resistant germplasm, the NB-LRR (nucleotide binding-site leucine-rich repeat) resistance genes can be rapidly identified and cloned using gene-targeted, resistance gene enrichment and sequencing method (Jupe et al. 2013; Witek et al. 2016).
Durability of quantitative resistance will, however, continue to depend on the size of the cultivation area of a variety as well as the dynamics of the pathogen population.
Phytophthora infestans Populations
Knowledge on the local pathogen population structure is important for the design of impactful disease management actions (Fry et al. 2015). In recent years, the initiatives EuroBlight (http://euroblight.net/), USABlight (http://www.usablight.org/), and TizonLatino (https://tizonlatino.github.io/) have been carrying out is monitoring of P. infestans populations.
This work has confirmed that P. infestans populations are constantly evolving and novel usually more aggressive genotypes appear periodically replacing the previously dominating genotypes. New genotypes can emerge through divergence from other genotypes, through recombination, or migration from other areas (Knaus et al. 2016).
The main mode of reproduction of P. infestans is asexual and variable numbers of lineages exist in different countries and regions. Several studies have confirmed that appearance of new genotypes can often be attributed to migration (Fry et al. 2015; Knaus et al. 2016; Saville et al. 2016).
Until recently the mating type A1 was dominating worldwide, except in the presumed center of origin, Mexico, where both mating types were found in similar frequencies (Goodwin et al. 1992).
This situation has changed dramatically, and A2 has now been reported in Scandinavia and Estonia (Hermansen et al. 2000; Runno-Paurson et al. 2016; Montes et al. 2016), Central Europe (Flier et al. 2007; Li et al. 2012b; Mariette et al. 2016), China (Zhu et al. 2015), Bolivia, Argentina, Uruguay and Brazil (Plata 1998; Deahl et al. 2003; Forbes et al. 1998; Casa-Coila et al. 2017), the USA (Rojas and Kirk 2016), Tunisia (Harbaoui et al. 2014), Algeria (Rekad et al. 2017), India (Chowdappa et al. 2015), and Canada (Danies et al. 2014).
However, even though both mating types are present in most cases, no evidence of frequent sexual reproduction has been found, suggesting that the sexual populations are ephemeral (Fry et al. 2015).
There are notable exceptions however, such as the Nordic countries, where it was shown that the sexual reproduction in the field is frequent and the oospores surviving in the field over winter in plant debris has led to earlier onset of epidemics (e.g., Widmark et al. 2007).
The diversity of P. infestans in South and Central America is a particularly interesting question, because these regions are extremely rich in biodiversity of Solanaceous species that are potential alternative hosts of this pathogen and thus can harbor divergent genotypes.
Furthermore, the centers of origin of the economically most important hosts, potato and tomato, are there. Interestingly, in South America, no sexual reproduction of P. infestans has been reported, and populations maintain strictly clonal structures.
In Colombia, Chile, Ecuador, and Peru the A1 mating type has been found mostly (Acuna et al. 2012; Perez et al. 2001; Forbes et al. 1997; Cardenas et al. 2011). In Mexico, in contrast, recombination is frequent and the population is extremely divergent with subdivisions associated with geographic regions (Wang et al. 2017b).
Mexico was also shown to be the origin of the current genotypes found in South America and continues to play an important role as the source population of the newly emerged aggressive genotypes in the USA (Saville et al. 2016; Goss et al. 2014).
Although P. infestans is generally heterothallic requiring two different mating types to form sexual oospores (image below), some isolates are homothallic. Recent studies have shown that these self-fertile isolates are found more frequently, constituting a new threat to potato and tomato crops because of their increased genotypic variability, better fitness, and greater aggressiveness (Zhu et al. 2016; Casa-Coila et al. 2017).
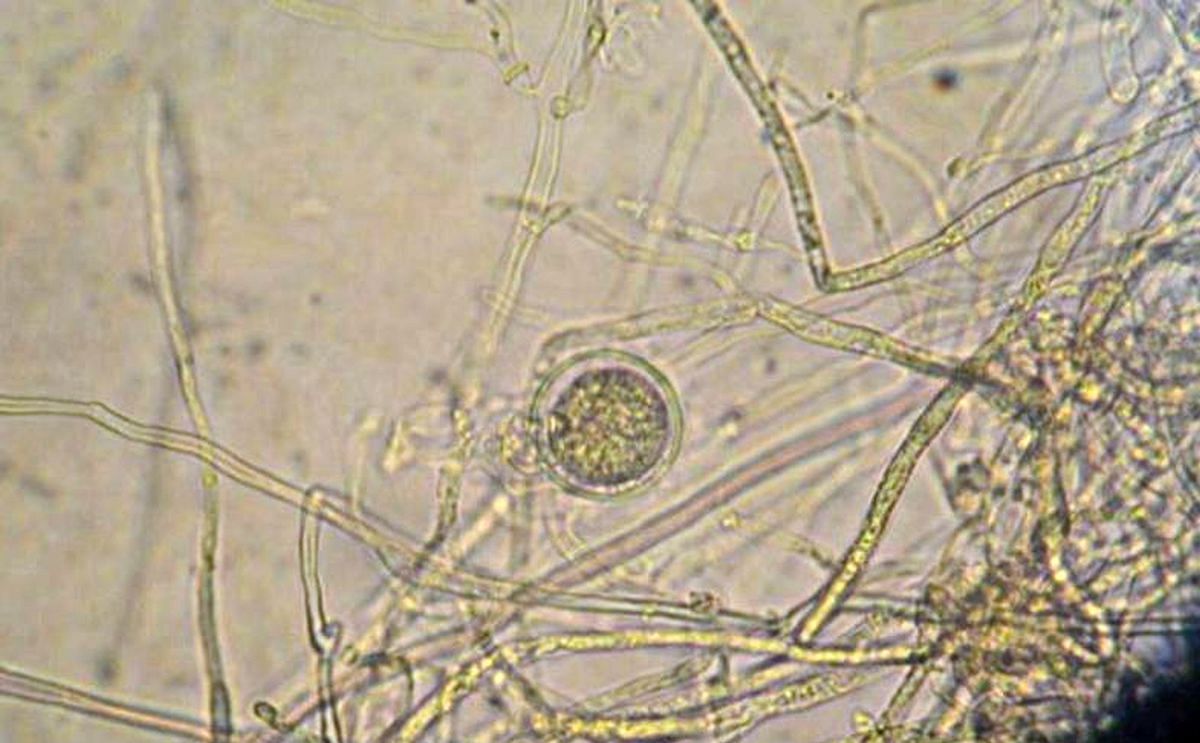
Oospore of Phytophthora infestans
Late blight of potatoes can be suppressed by a combination of approaches. As a polycyclic disease that explodes under favorable conditions, integrated strategies are crucial. These include sanitation measures that eliminate or reduce initial sources of the disease (e.g. infected seed, cull piles, infected neighboring plots, and volunteers), prophylactic fungicide sprays before the appearance of symptoms, curative fungicide sprays and use of resistant cultivars to reduce the rate of disease development, use of early-maturing cultivars to reduce the duration of the epidemic, or planting the crop in seasons or areas where the environment is not favorable for the pathogen.
The application of chemical fungicides continues to be the most common strategy for late blight control, making late blight one of the top drivers for pesticide use in the world. The demand for weekly applications generates a billion-dollar business globally every year (Haverkort et al. 2009). To optimize the use of fungicides, it is important to know the efficacy and type of activity of the active ingredients.
The frequency and timing of fungicide applications may depend on the foliar resistance of the cultivar, fungicide characteristics, rate of growth of new foliage, weather conditions, irrigation, and incidence of blight in the region (Cooke et al. 2011).
The range of fungicide types available to farmers vary depending on the numbers of products registered and commercialized in their area. In Europe the number of registered products is being reduced due to health and environmental concerns and in some European countries farmers have access to less than ten fungicide products for late blight control (http://www.endure-network.eu). In other countries the process of registration of pesticides is less restricted.
For example, in Ecuador, hundreds of fungicide products are registered for late blight control based on more than 30 active ingredients of which most contain old generic substances like mancozeb, cymoxanil, and carbendazim (http://www.agrocalidad.gob.ec), yet in other countries only a couple or so products are readily available to farmers due to trade limitations.
The most efficient and arguably the most elegant strategy to control late blight is the use of host resistance. Today, it is well known that with the use of genetic resistance late blight can be controlled with less fungicide either by lowering the fungicide dose or using longer application intervals (Kirk et al. 2005; Nærstad et al. 2007; Cooke et al. 2011; Liljeroth et al. 2016; Haverkort et al. 2016).
The use of resistant varieties could sharply reduce losses from late blight, especially in developing countries, where disease is less well managed for many reasons (e.g. high disease pressure, problems of access to fungicides, and inadequate farmer knowledge of disease dynamics).
Nevertheless, the use of resistant varieties continues to be an uncommon disease management approach as susceptible varieties are promoted and required by many wholesalers and processing industries, leaving farmers with little option but to grow susceptible varieties (Forbes 2012).
Integrating genetic resistance and chemical control helps in reducing the use of fungicides, decreases production costs, and reduces damage to human health and the environment (Perez and Forbes 2010; Cooke et al. 2011).
One way of achieving better design and integration of management elements is through the use of simulation models (reviewed by Forbes et al. 2008) and especially decision support systems (DSS). These typically integrate and organize all available information on the life cycle of P. infestans, monitoring of inoculum, weather (historical and forecast), plant growth, fungicide characteristics, cultivar resistance, and thereby predict disease pressure and action thresholds that can guide decision-making.
Based on the information provided by the DSS farmers can make informed disease management decisions.
DSS can deliver general or very site-specific information to the users via extension officers, telephone, SMS, e-mail, and websites (Cooke et al. 2011). In the case of smallholders in developing countries, basic information to understand the disease is critical to improve management (Nelson et al. 2001; Andrade-Piedra et al. 2009; Ortiz et al. 2019).
Cultural control involves all the activities carried out during agronomic management which alter the microclimate, host condition, and pathogen behavior in such a way that they avoid or reduce pathogen activity (survival, dispersal, and reproduction) (Garrett and Dendy 2001).
Among them are the elimination of volunteers and cull piles and associated debris, use of clean seed potatoes preferably certified seed, use of resistant varieties, adequate space between rows and plants, rotation with other crops not susceptible to late blight, adequate hilling, harvest in dry conditions and when the tubes are mature (Garrett and Dendy 2001; Perez and Forbes 2010).
Mixtures of potato varieties (resistant and susceptible) offer partial improvement on disease suppression (Phillips et al. 2005; Pilet et al. 2006). Under temperate climate conditions and where tuber infections are a concern, potato vines are typically killed by applying chemical desiccants 2–3 weeks before harvest (Perez and Forbes 2010).
Biological control consists of reducing disease through the interaction of one or more live organisms with the disease-causing pathogen or use of extract of plants. Some findings report the use of Trichoderma isolates (Yao et al. 2016), Chaetomium globosum (Shanthiyaa et al. 2013), Trichoderma viride and Pennicillium viridicatum (Gupta 2016) and bacteria from the genera Bacillus, Pseudomonas, Rahnella, and Serratia (Daayf et al. 2003) as biocontrol agents in the management of late blight disease in potato.
Garlic has, for example, been suggested as a potential intercropping plant for the management of potato late blight disease under Ethiopian condition (Kassa and Sommartya 2006). Still, few biological control measures are used by nonorganic growers due to low efficacy and farmers’ lack of knowledge about these options and access to the most efficient products.
Looking Forward
Phytophthora infestans has proven capable of overcoming fungicides and resistant varieties through decades. Potato late blight, thus, continues to be the main potato constraint worldwide despite huge investments in its management. The growing intercontinental trade in potato is also increasing the risk of worldwide dissemination of dominant P. infestans strains.
The threat from the disease will without doubt continue in the future. Fortunately, encouraging solutions to improve its management are arising from new advances in molecular, sensor, computational, and smartphone technologies.
Efficient inoculum monitoring tools are becoming more accessible that can indicate whether P. infestans is in an area for the guidance of the initial fungicide spray and P. infestans population’s movements (Fall et al. 2015). These could even be connected to molecular diagnostics that can predict phenotypic traits of the pathogen population, such as fungicide sensitivity and R-gene interaction, and perhaps even predictions of aggressiveness and fitness in a matter of hours.
Spore traps could eventually be mounted on tractors or drones allowing for real-time spatial monitoring (Fall et al. 2015; Fry 2016). New high-throughput methods for monitoring and assessing plant populations are being developed, such as remote sensing, image processing, and web-based farmer-extension service networks.
Such indirect disease detection approaches are improving disease surveillance significantly with high potential in remote and low-input production systems. Smartphone-based extension systems linked to rapid and portable molecular diagnostics kits (e.g. Loop-mediated isothermal amplification—LAMP—and lateral flow immunodiagnostics tools) can support immediate dissemination of disease information to a large number of farmers.
If made widely available such smartphone tools, hand-held portable diagnostics kits and novel weather sensors could lead to precision management and significant impacts on production capacity. Farmer-extension service networks based on internet connectivity are already important options that improve disease surveillance and supplying fast access to appropriate and up-to-date knowledge on pathogen distribution and management (Fry 2016).
Capacity building (for farmers and extension services) to improve disease management skills, coupled to early-maturing and resistant cultivars, novel diagnostic tools, improved DSSs, and low-toxicity fungicides have the potential to reduce crop loss, management costs, and environmental impact, and even more so as biological options become accessible to farmers.
Current research opportunities in late blight management focus on P. infestans studies, fungicide testing, dissemination of resistant cultivars and validation of DSSs and training. In addition to continued research and extension efforts, alliances with the agro-chemical industry seem to be necessary to fully achieve integrated pest management strategies (Pacilly et al. 2016).
This is being promoted through local and regional research networks that via collaboration strengthen institutional capacity in research and extension related to late blight (see http://euroblight.net/). Emerging research topics on P. infestans include the role of the pathogen–microbiota interaction in promotion or suppression of the disease (Larousse and Galiana 2017) as well as the metabolism of P. infestans (Judelson 2017), its effector repertoire on the plant, and, in fine, how it promotes or suppresses the disease.
At the same time, P. infestans as an infectious entity is no longer only considered at the species or lineage level but also at the level of a resident microbiota or part thereof (Larousse and Galiana 2017).